Our group focuses on experimental and theoretical atomic, molecular and optical (AMO) physics. On the experimental side, we develop novel experimental technologies (this includes lasers, cryogenic and vacuum technologies, precision mechanics, digital and analog electronics, etc.) with the primary goal of studying and testing quantum physics for atomic and molecular systems. On the theory side, we work on a wide range of AMO-physics problems ranging from quantum-scattering calculation, molecular property calculations, up to populating spectroscopic databases, with the primary goal of supporting our experimental endeavors.
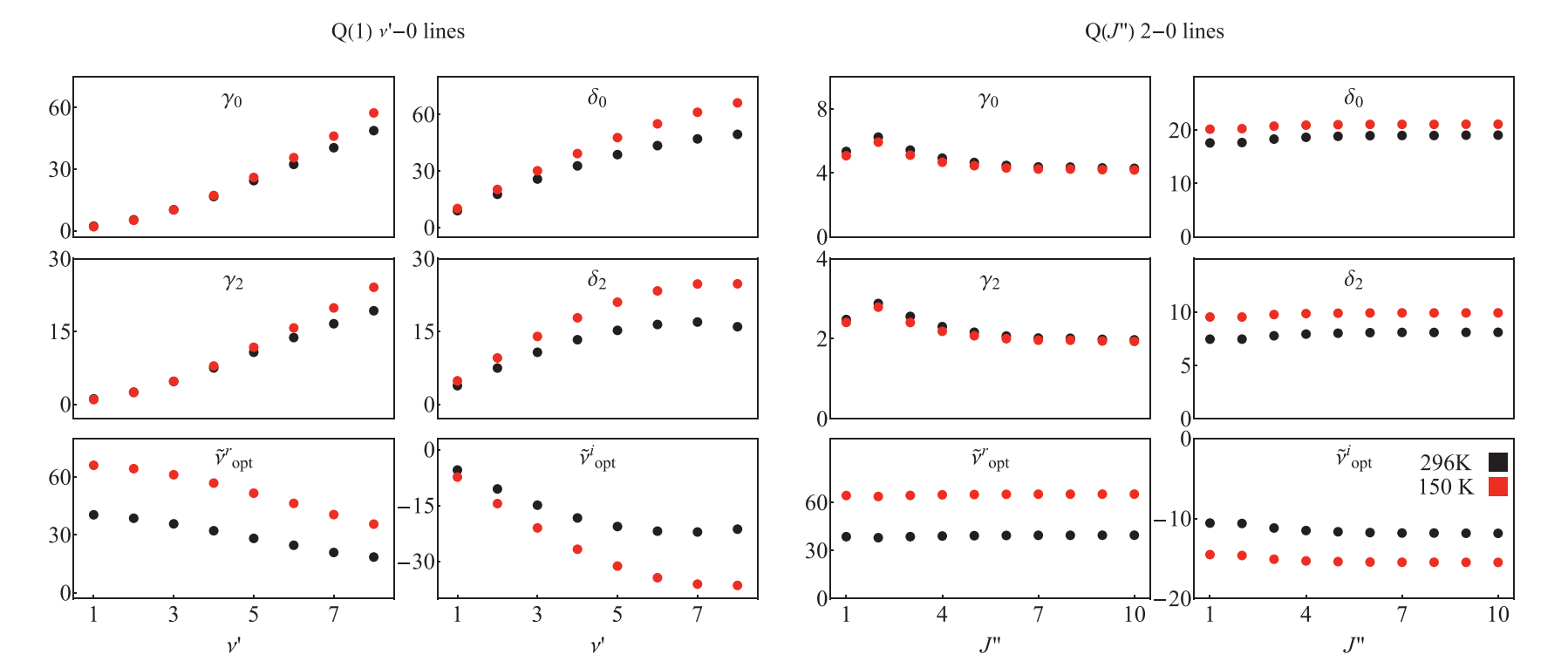
An excerpt from our dataset illustrating the structure of the dataset and the examples of the vibrational and rotational dependences of all six line-shape parameters. The line-shape parameters are determined for the He-perturbed H2 rovibrational lines at T = 150 and 296 K; refer to red and black colors, respectively. All the parameters are expressed in units of 10−3 cm−1atm−1. The values of the line-shape parameters shown in this plot are not directly taken from ab initio calculations, but reconstructed from the DPL relations, Eqs. (7), based on the coefficients from our dataset [34]. (For interpretation of the references to color in this figure legend, the reader is referred to the web version of this article.)
Logotypy
Lorem ipsum dolor sit amet, consectetur adipiscing elit. Ut elit tellus, luctus nec ullamcorper mattis, pulvinar dapibus leo. Lorem ipsum dolor sit amet, consectetur adipiscing elit. Ut elit tellus, luctus nec ullamcorper mattis, pulvinar dapibus leo. Lorem ipsum dolor sit amet, consectetur adipiscing elit. Ut elit tellus, luctus nec ullamcorper mattis, pulvinar dapibus leo. Lorem ipsum dolor sit amet, consectetur adipiscing elit. Ut elit tellus, luctus nec ullamcorper mattis, pulvinar dapibus leo. Lorem ipsum dolor sit amet, consectetur adipiscing elit. Ut elit tellus, luctus nec ullamcorper mattis, pulvinar dapibus leo.